The Carter lab is interested in a family of microtubule motor proteins called dyneins. We focus on cytoplasmic dynein: a transporter that moves cargos throughout our cells. We use structural biology (cryo-EM and X-ray crystallography), in vitro reconstitution, single molecule assays, and many other complementary approaches to ask how the motor moves, how it is regulated and how it can carry so many different objects.
Highlights include: X-ray crystal structures of the dynein motor domain that explain how it couples ATP hydrolysis to movement; the discovery that certain cargo adaptors activate dynein’s long-distance movement; cryoEM structures of dynein and its co-factor dynactin that reveal how dynein is activated.
A key feature of cytoplasmic dynein is the large number of roles it plays in the cell. It transports a large array of different membrane organelles and everything from aggregated proteins to viruses. It also has critical roles in cell division. The lab’s current focus is to work out what components are required to recruit dynein to specific cargos and how the transport is controlled.
The dynein motor
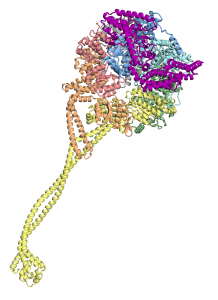
All dyneins contain a motor domain made of a ring of six AAA+ domains. The AAA+ family are a widespread class of ATPases that typically unfold or remodel other proteins. A major question was how dynein uses its AAA+ architecture to generate force toward the minus ends of microtubules. Work from Andrew and the lab showed how dynein binds microtubules (Carter et al 2008, Lacey et al 2019) and determined the architecture of the motor domain (Carter 2011, Schmidt 2012).
A key result was an X-ray crystal structure of a dynein motor in its ATP hydrolysis conformation (Schmidt et al 2015). This showed how changes in the AAA+ ring bend a rod-like linker domain, priming it to generate force. It also addressed how the AAA+ ring communicates to dynein’s microtubule binding domain (MTBD) via a long range sliding motion within the coiled coil stalk that connects them.
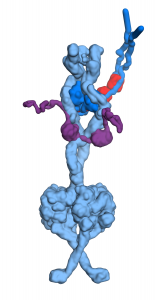
In 2017 we reported a cryo-EM structure of full length cytoplasmic dynein in an inhibited state called the phi-particle. The two dynein heavy chains bind to accessory chains and wrap around each other to form the tail region before joining into the motor domains. Our structure showed how the packing of the motor domains locks them into a state that is unable to bind microtubules.
In a collaboration with Ahmet Yildiz’s lab we addressed the basis of dynein’s directionality. We mutated the dynein stalk and used cryo-EM to show that this reversed the direction the motor points when bound to microtubules. This engineered motor moved robustly in the opposite direction to wild-type dynein showing that the angle of the stalk is a major determinant of directionality (Can et al 2019).
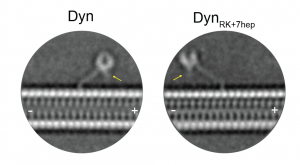
Connecting dynein to cargos
Our first work on cargos focused on BICD2, an adaptor known to link dynein to Golgi vesicles. We made fully recombinant human dynein and discovered that both BICD2 and dynactin are required for it to move over long distances (Schlager et al 2014). This unanticipated finding established the principle that cargo adaptors can activate dynein and suggested a simple way in which dynein is controlled by the cargo it carries.
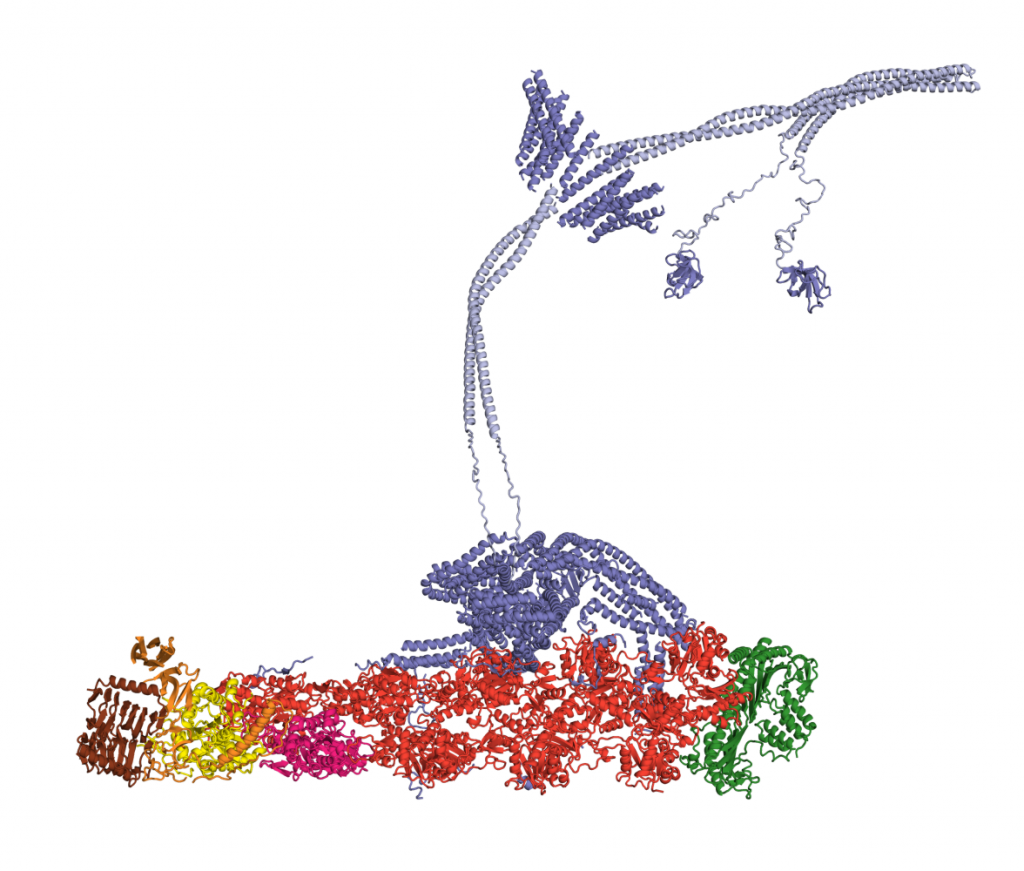
In early 2015 we published cryoEM structures of dynein’s essential cofactor dynactin: a 23 subunit complex built around a filament of the actin relate protein Arp1. The work revealed the architecture of dynactin and explained how the length of the Arp1 filament is specified to be a defined length. We also addressed how dynactin binds dynein. Instead of forming a loosely coupled complex, as previously anticipated, we showed that dynein and dynactin form an intimate interaction with BICD2 acting as the glue that sticks the dynein tail to the dynactin filament (Urnavicius et al 2015). Subsequently we used EM to uncover how this interaction drives large rearrangements in dynein and how this activates its long distance movement (Zhang et al 2017).
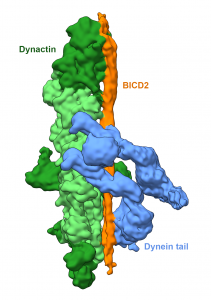
BICD2 is one of a growing family of cargo adaptors that can activate dynein movement. These adaptors contain a long (>200 amino acid) coiled coil but lack conserved sequence motifs. In 2018 we published cryoEM structures of dynein/dynactin bound to two new adaptors: BICD-related-1 (BICDR1) and HOOK3 (Urnavicius et al 2018). These show both adaptors robustly recruit a second dynein to dynactin. The two dyneins lie side-by-side stabilizing them sufficiently to allow the determination of a high-resolution structure of the dynein tail. We also used single molecule motility assays to show that the two dyneins made the dynein/dynactin complex significantly faster than when a single dynein is present.
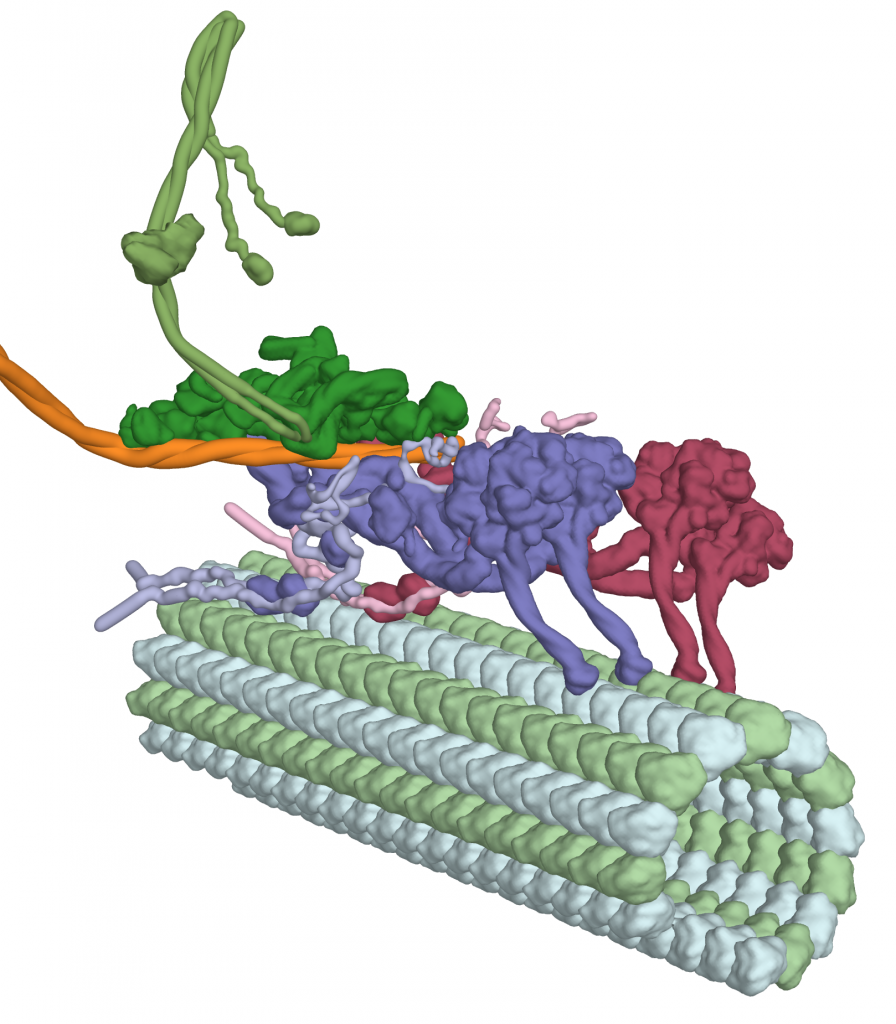
Current Projects
Part of our current focus is on how the core dynein/dynactin/adaptor machinery connects to the large number of different cargos it transports in cells. Our interests include different membrane bound organelles, RNAs (in collaboration with the Bullock lab) and viruses. Our approach involves structure determination, cell biology approaches, in vitro reconstitution of dynein/cargo complexes, as well as direct visualization of cargos inside the axons of neurons by cryo-electron tomography. The lab also works on projects related to dyneins found in cilia and flagella.